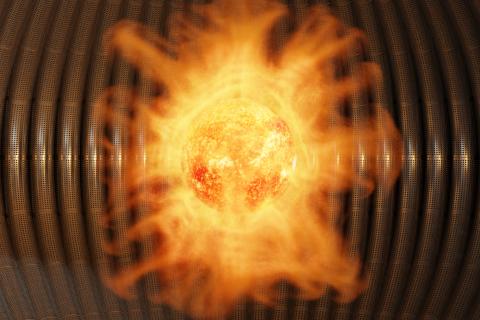
Nuclear fusion: steps towards energy production
(This article was originally published in L'Édition N.20)
As stars do, several experimental devices are able to initiate nuclear fusion reactions, which could be a source of colossal, low-carbon and safe energy, and the first steps towards future fusion reactors. Scientists at Université Paris-Saclay are involved in this research.
Initiated by the Covid-19 pandemic and brought to a head by the war in Ukraine, the current energy crisis is revealing truths that have long been ignored. It confirms the urgency of breaking our dependence on fossil fuels and the need for a transition to low-carbon energies (renewable and nuclear) in line with the fight against global warming.
At the same time, this crisis shows how important the world’s energy needs are and the calls for energy sobriety underline how difficult it is to reduce these needs without efforts and profound changes. Moving away from fossil fuels to low-carbon energies is not easy, given their large share of the energy mix. Moreover, despite their many advantages, none of these energies is alone fully meeting the energy needs of the current societies. As a result, there is little hope of offsetting fossil fuels with a single source of low-carbon energy that is at the same time unlimited, clean, safe and affordable.
Merging nuclei for energy
One that raises hopes for the longer term is nuclear fusion, which is the opposite of nuclear fission, the process used by currently operating nuclear power plants to produce energy from atomic nuclei. Like what happens at the heart of stars, nuclear fusion reactions bring light atoms together to form slightly heavier atoms and release energy at the same time.
The most studied and promising reaction for energy production is the fusion of two variants, or isotopes, of hydrogen (deuterium, D, and tritium, T) to give one helium atom (alpha particle), one neutron and a large amount of energy. For the same mass, this energy is four times greater than that obtained by nuclear fission, and four million times greater than that released when coal, oil or natural gas are burned. Only a few hundred micrograms of fuel are needed for this. Moreover, no runaway reaction would be possible here. Another advantage is that deuterium is naturally present in large quantities in the oceans and tritium, which is available in smaller quantities, can be produced from lithium, which is abundant in nature. Nuclear fusion would also cause fewer waste management problems.
Unique conditions, to be reproduced
This process seems to have nothing but advantages. Yes, but it also requires a lot of effort... To merge atomic nuclei, they must be close together. This means overcoming their mutual electrical repulsion. In a star, the temperature – over 10 million degrees Celsius – lifts this barrier. Matter is in a plasma state, different from the solid, liquid and gas states. In a plasma, electrons (negatively charged) are torn from the atoms and no longer orbit the nuclei, which are ionised (positively charged). The extreme pressures found at the heart of stars confine the nuclei to a small space, which increases the probability of their collision, and therefore their fusion.
On Earth, such conditions do not exist naturally. Other means have to be used to reproduce them. Three conditions are necessary to make nuclear fusion an abundant and profitable source of energy. In addition to heating the fuel to a temperature ten times higher than in stars and triggering the agitation and collision of the plasma nuclei, it is necessary to confine the plasma to achieve a sufficient density of nuclei and increase the probability of collision. Above all, it is essential to maintain the stability of the plasma and its confinement for a sufficient time to extract energy. Currently, two confinement methods are being developed: magnetic confinement and laser inertial confinement.
Inertial confinement fusion: the impact of the laser
On 5 December 2022, a major first was achieved by inertial confinement fusion. The 192 ultra-powerful laser beams of the National Ignition Facility (NIF) at Lawrence Livermore National Laboratory (USA), aimed at a gold cylinder containing a deuterium-tritium target enclosed in a bead (a so-called indirect attack scheme), succeeded in triggering a nuclear fusion reaction that delivered 3,15 megajoules of thermonuclear energy from a laser pulse of 2,1 megajoules, i.e. an energy gain of 1.5. “This shot has boosted interest in inertial fusion energy, even though there are no plans to develop a fusion reactor for the time being,” points out Benoît Canaud, from the Matter under Extreme Conditions Laboratory (LMCE – Univ. Paris-Saclay, CEA). This achievement must not eclipse the many challenges that still need to be met in order to develop a commercial solution based on inertial fusion: increasing the efficiency and rate of the lasers, increasing the energy produced by each experiment and lowering the manufacturing cost of the targets.
At the LMCE, Benoît Canaud and his colleagues are studying another laser attack scheme than that of the NIF. This is a direct attack. “We use a very high-energy laser to illuminate a target (a microbead) of cryogenic DT (at a temperature of 20 K, i.e. -253.15 °C). This sets the target in centripetal motion so that it collapses on itself.” The laser heats the outer layer of the target (shell) to several million degrees Celsius (over 1,000 eV or 1 keV) making it spray as plasma. “The ablator, made of plastic, is expelled at very high speed and as it expands outwards from the shell, it pushes towards the interior of the target. This sets the cryogenic layer in motion, which gradually implodes until it collapses in the centre. As the cryogenic layer is increasingly compressed in the centre, the DT in the form of residual gas sees its temperature rise to around ten keV.”
The way the laser hits the target is important. “In inertial confinement fusion, we generate shocks of varying strength inside the ablator and the cryogenic DT. A shock dumps entropy (disorder) and increases the material’s internal energy, which makes it much harder to compress.” The art of inertial confinement fusion is therefore to make the target implode without putting too much entropy into the cryogenic DT to be compressed, in order to produce a high thermonuclear gain. “The more we compress the target – that is to say, we reduce the volume – the more the density and power gain increase.”
Magnetic confinement fusion: the tokamak device
Whereas inertial confinement fusion uses very short times, around 100 picoseconds (0.1 ns), in order to inertially confine the fusible matter to very high densities, magnetic confinement fusion uses long times and relatively low particle densities. Tokamaks or stellarators are currently the most advanced systems for future reactors. These toroidal or annular chambers are equipped with a fuel heating system and magnetic coils through which an electric current flows. As any charged particle in a magnetic field wraps around the field lines, ions and electrons in the plasma are magnetically confined in the chamber by the powerful magnets.
Some of these experimental devices show a few records, although none has yet managed to achieve a net power gain of more than 1, i.e. to produce more energy than that required to heat the plasma and confine it. At the end of 2003, the French tokamak Tore Supra – renamed WEST for Tungsten (W) Environment in Steady-state Tokamak and located at the CEA in Cadarache – maintained its plasma for six minutes and thirty seconds. At the end of 2021, the Chinese HL-2M tokamak did so for seventeen minutes and thirty-six seconds. The English tokamak JET (Joint European Torus), supported by the EUROfusion consortium comprising European and Swiss research institutes, has achieved a ratio of 0.67: by 1997, it produced 16 megawatts of fusion power for a total heating power of 24 megawatts. At the beginning of 2022, it delivered 59 megajoules of fusion energy for five seconds.
From experiment to industrial demonstrator: ITER
The missing link between these experimental devices and future industrial demonstrators is the international thermonuclear experimental reactor ITER in Cadarache, an ambitious project involving 35 countries. It must demonstrate the scientific feasibility of magnetic confinement fusion to produce electricity and the safety of the device. The start of operations and the production of the first plasma are expected in 2030. ITER will produce a deuterium-tritium plasma heated to over 150 million degrees Celsius and self-sustaining by the fusion reactions. The tritium used in the reaction will be regenerated within the chamber from the neutrons released during the reaction. For 400 seconds, ITER will deliver 500 megawatts of thermal power from an injected power of 50 megawatts, which means a gain of ten. To achieve this, the volume of its chamber (1 400 m3) and its plasma (840 m3) will be much larger than those of the current tokamaks.
Several laboratories of Université Paris-Saclay are part of the EUROfusion consortium and contribute to ITER through their research. The Laboratory of Plasma Physics (LPP – Univ. Paris-Saclay, CNRS, École Polytechnique, Observatoire de Paris, Sorbonne Univ.), whose expertise lies in the diagnosis of plasma fluctuation and turbulence, is one of them.
When turbulence comes to the plasma
“An extremely hot and magnetised plasma is chaotic and complex. There is a zoology of instabilities due to gradients in temperature, density, average speed, current, magnetic field, etc.,” explains Pierre Morel, from the LPP’s Magnetic Fusion team. “The temperature at the centre of the plasma is thus several hundred million degrees Celsius, while a few metres further on, the superconducting magnets are bathed in liquid helium, which is close to 0 K (-273,15 °C). The magnetic field is not homogeneous either: it is more intense inside the ring than outside. This is a source of instabilities, which grow exponentially and coexist on various scales in the plasma.”
As they cannot grow indefinitely, these instabilities saturate and lead to turbulence, which is characterised by vortices that cascade and fracture into smaller vortices until they dissipate. These vortices can also coalesce and create larger structures. As the turbulence is predominantly perpendicular to the magnetic field, these structures end up mixing the hot particles in the centre of the plasma with the outer, colder ones. On the contrary, other phenomena have a beneficial effect on the turbulence, such as zonal flows that shear the vortices. “We are studying how the structures that predominate in the mixture are formed, what their behaviour, their size and their life time are. Our aim is to maintain high heat and a high density of particles in the centre of the magnetised plasma in order to keep the plasma confined for as long as possible,” explains Pierre Morel.
Because of the mixing, the quality of the energy and particle confinement deteriorates. “The plasma confinement time, i.e. the average time during which the energy remains inside the system, is fixed by the turbulence. It can be increased by multiplying the layers of magnetic lines. As a result, less external power is needed to maintain the reaction. This is why we are developing a large machine like ITER,” says Pascale Hennequin, head of the LPP’s Magnetic Fusion team.
Diagnostics and numerical simulations: paving the way for ITER
The team is developing diagnostics that are implemented in different tokamaks, such as WEST, ASDEX Upgrade in Germany and TCV (Variable Confinement Tokamak) in Lausanne (Switzerland). They measure the turbulence rate of the plasma. The aim is to compare the different machines and their parameters. “The purpose is to validate the new operating regimes in order to make the predictions of ITER and future reactors more reliable,” says Pascale Hennequin. All observations are made by indirect measurements. “We probe different parts of the plasma by scattering micrometric electromagnetic waves. It is a bit like a radar. We measure the speed of the density fluctuation of the particles at different scales. This speed plays a very important role in all the saturation and turbulence regulation processes.”
The team compares these observations with its numerical simulations modelling turbulence and the appearance and characteristics of instabilities. “We use a kinetic description: we model the way in which the particles are distributed in speed and position within the plasma. Our aim is to produce simulations that faithfully and realistically represent what we see in our experiments and diagnostics.” By extrapolating these results, scientists are seeking to provide reliable simulations before operating the ITER tokamak and to predict its confinement time.
Although process optimisation and a finer understanding of the physics have yet to be achieved, the barriers surrounding nuclear fusion energy production are gradually coming down and a potential industrial future is looming. Proof of this is the growing emergence of start-ups collaborating with research centres and universities to develop the activity.
Publications
- Mauro Temporal, Benoit Canaud, and Rafael Ramis. Dependence of Inertial Confinement Fusion capsule performance on fuel reaction rate, Eur. Phys. J. D (2021).
- U. Stroth et al. Progress from ASDEX Upgrade experiments in preparing the physics basis of ITER operation and DEMO scenario development. Nucl. Fusion 62, 042006, (2022).