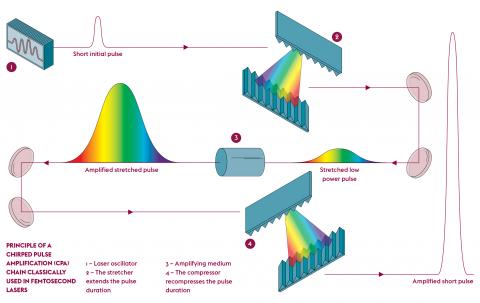
When light is brief but intense
(This article was originally published in L'Édition n°16)
Scientists at Université Paris- Saclay are shedding light on the phenomena and uses of femtosecond lasers. These devices, with their ultrashort pulses and ever-increasing power, use a unique kind of radiation.
Few inventions have had such a successful history as the laser. Conceived in theory in 1917 by Albert Einstein, the first amplified, coherent, monochromatic radiation device appeared in 1960, thanks to the work of the American physicist Theodore Maiman. Since then, the device has become widely popular and has undergone many improvements. It is now indispensable in fields as varied as medicine, telecommunications, imaging and micromachining. New types of lasers have emerged. Their features, including the spectral width of emission, pulse duration and rate, energy, power and power density delivered, open up new horizons.
The goal is to generate very high intensity ultra-short pulses compatible with new applications and the study of biological, physical or chemical phenomena taking place on extremely short time scales, like those explored by researchers at Université Paris-Saclay. Popularised in the 1990s thanks to their use in eye surgery, femtosecond lasers are leading the way. They now deliver pulses of ten to several hundred femtoseconds (fs, 10-15 s) with units of power reaching a terawatt (TW, 1012 W) or even a petawatt (PW, 1015 W).
A high-performance laser amplification chain
Several lasers are available for use in the Interactions, Dynamics and Lasers Laboratory (LIDYL – Univ.Paris-Saclay, CEA, CNRS). Among these, those from the EquipEx ATTOLAB (classified as an ‘equipped with excellence’ facility) routinely produce perfectly controlled pulses of 15 mJ at a high frequency and with a duration of about 23 fs. This duration can be reduced to 17 fs by adjusting the settings.
“The particularity of the ATTOLAB Orme platform managed by LIDYL is that it offers a dual-frequency (1 and 10 KHz) laser amplification chain (FAB 1-10) with infrared emissions and attosecond secondary sources (as, 10-18 s) emitting in the extreme XUV range,” says Jean-François Hergott from the Support and Short Pulse Lasers team (SLIC). FAB 1-10 is the result of work started over ten years ago with the company Amplitudes Technologies, as part of the Impulse joint laboratory. “The entire amplification chain has been designed for greater stability. The laser’s surroundings have been improved and the facilities upgraded.” The team focused in particular on stabilising the first stage of the chain using a more efficient regenerative cavity which is compatible with higher speeds, which in turn cause higher thermal loads. Combined with an acousto-optic tunable filter, the whole system improves optical efficiency and delivers three times more energy at output than a ‘classic’ arrangement. Another development has been the precise control of the position of the oscillation field in the time window for the amplified pulse.
The team is now continuing to reduce pulse length. “A high-energy post-compression system has been developed. This is an intermediate step between the laser output and the generation of XUV radiation. In a hollow optical fibre drawn in a vacuum, the laser pulse is coupled with gas. After nonlinear interaction, pulses of 3.9 fs and 2.5 mJ are obtained.” By connecting these pulses to the platform’s XUV stations, the team aims to generate unique attosecond pulses, with which they can study and control electron dynamics in matter.
Observing nanoscale objects on attosecond time scales
This system, known as strong field, is at the heart of the research carried out at LIDYL by Hamed Merdji and Willem Boutu. They are driven by the understanding of ultrafast phenomena at the nanoscale and are particularly interested in the new area of petahertz electronics. “In electronics, today’s processors operate at frequencies in the gigahertz (GHz, 106 Hz) range. The petahertz frequency (PHz, 1015 Hz) would make it possible to respond to new calculation and information storage problems. This requires new methods to track the movement of electrons in semiconductors at the nanoscale and attosecond time scales,” points out Hamed Merdji.
The physicists rely in particular on coherent diffraction imaging (CDI) which consists of illuminating an object with coherent light to collect the diffraction pattern generated and then reconstruct the phase encoded on the hologram and the object’s image, using inversion algorithms. “The principle is similar to what we observe with sunlight when it passes through a hole in a leaf on a tree. The light rays are diffracted and spread out in several directions. The angle they take conveys information about the size of the hole. The smaller the hole, the larger the angle of diffraction. With a camera, the image of the passage of light is recorded and by using coherent light, the radiation phase is encoded, which conveys spatial information about the object.”
In the work carried out, CDI uses coherent XUV or ultra-short X-ray radiation. “If you want to observe nanoscale objects, you need light sources with a nanoscale wavelength.” However, in the case of attosecond pulses, the extremely broad spectrum of these XUV light sources blurs the diffraction image obtained in CDI. “Each of its wavelengths produces a diffraction image and they all overlap in an inconsistent way. As a result, the encoded information is lost.”
To “disentangle” them, the team has developed a unique algorithm which uses an inversion system based on mathematical projections of the spectrum. The resulting “de-blurring” produces an average diffraction pattern which can be analysed by CDI reconstruction algorithms. The team validated its method using coherent X-rays from the NANOSCOPIUM beamline of the SOLEIL synchrotron. “The theoretical spectral content of an attosecond pulse was experimentally reconstructed.” In the near future, the plan is to apply the mathematical tool to a system involving a real attosecond source.
Functionalisation of transparent materials
At the Orsay Institute of Molecular Chemistry and Materials (ICMMO – Univ.Paris-Saclay, CNRS), the team led by Bertrand Poumellec and Matthieu Lancry promotes the use of femtosecond lasers as a unique tool for the in-depth modification of transparent materials (glass, optical fibre, film, solid or vitreous substrate, polymer and crystal) and for implementing a whole series of functions (micromechanical, optical, microfluidic, etc.), regardless of their shape and composition. Once they have been functionalised, the objects become sensitive to the environment and are used as sensors for temperature, pressure, deformation, concentration, etc. “The laser reduces the number of manufacturing methods. lt avoids assembly and gluing, minimises errors and improves the lifetime of objects,” explains Matthieu Lancry.
“All materials, even transparent ones, are sensitive to light intensity,” points out the researcher. With pulses of 0.1 μJ to a few μJ and of the order of TW/cm2, the radiation no longer passes through the medium but is absorbed over a volume of a few μm3. With it, the local density and the refractive index of the material changes. “Light is a bit like a hand which manages to sculpt a material without touching it,” suggests Bertrand Poumellec.
Everything happens at the interface between the light beam and the plasma produced by the material. Phase transitions occur, such as the formation of nanobubbles (or nanopores) of about ten nanometres in diameter, or the creation of nanonetworks. While density changes are not very thermally stable, refractive index changes can withstand very high temperatures. “It gives access to extreme environments, such as future generation nuclear power plants, aircraft engines and gas drilling sites, etc.” explains Matthieu Lancry.
Controlling the size of all these changes is of paramount importance. “For example, if the nanopores are too large, the signal spreads too far and is lost.” Smaller pores, which optical components find easier to deal with, cause less optical and scattering problems, but are also less thermally stable. By adjusting the laser settings, the researchers are able to alter their size. “The laser’s writing speed and the repetition rate are used to accumulate more or less heat in the material.” The challenge is to improve our understanding of writing and erasing mechanisms in order to stabilise the components in their future operating conditions. “It is important for manufacturers to have components which will not deviate and will remain reliable throughout their lifetime.”
In recent years, a new breakthrough has been achieved thanks to a model developed in the laboratory which predicts the erosion kinetics of nanopores according to their size and the material’s chemical composition. This expertise puts the ICMMO team in a good position to propose new ‘recipes’ for materials and obtain even more thermally stable sensors. The compositions focus on mixtures of 95% silica (SiO2) and 5% germanium oxide (GeO2), SiO2 and aluminium oxide (Al203), or SiO2 and zirconium oxide (ZrO2). “We are starting to see an effect for mixtures with more than 30% Al2O3.” The team is currently finalising a model which predicts the formation of nanopores and are developing new optical fibres from glass, semi-crystalline and glass-ceramic compounds for temperature conditions over 1,500 °C.
Terahertz waves for remote spectroscopy
At the Matter in Extreme Conditions laboratory (LMCE – Univ.Paris-Saclay, CEA), Luc Bergé, Laurent Gremillet and colleagues are interested in the generation of terahertz (THz) electromagnetic waves by femtosecond laser. Using particle numerical simulations (PIC), the team speculatively reproduces the physical parameters of target irradiation experiments in order to improve the conversion efficiency of the laser pulse into THz radiation and obtain the most energetic THz source possible. The challenge is successfully differentiating between the different sources of THz emissions.
At intensities above 1014 W/cm2, laser pulses almost instantaneously ionise the molecules of the irradiated target, transforming it into a plasma and inducing low-frequency electronic currents. These photocurrents are sources of THz emissions, which are even more effective as the oscillations of the laser electric field used are asymmetric. This is achieved by using multi-coloured laser pulses. When the laser intensity exceeds 1018 W/cm2, a fraction of the target’s electrons are accelerated to near-light speeds and a new, relativistic interaction regime is established. These electrons are at the origin of many radiative processes, including the transition coherent radiation emitted when the electrons cross the interface between the target and the vacuum.
These electron acceleration mechanisms and the associated THz emission processes depend on the nature of the target. If it is transparent, such as a low-density gas, the laser propagates in volume and the acceleration of the electrons to energies in the GeV range occurs indirectly. “The laser pulse ionises the gas and behind, a plasma wave develops in which the electrons are accelerated. When they escape from the plasma, there is radiation at the interface,” explains Laurent Gremillet. If the target is solid, “the laser is reflected and everything happens at the surface.” The electrons reach lower energies, around a few MeV, but their number is greater. Nevertheless, both configurations lead to intense transition radiation in the THz range.
Until recently, THz emission had applications in spectroscopy and materials characterisation. But new needs are emerging in selective chemistry, tomography and medicine. Others are emerging in remote spectroscopy. The ALTESSE project, which began in 2015 in collaboration with the Franco-German Research Institute of Saint-Louis and the Centre for Intense Lasers and Applications, was intended to prove the feasibility of coherent THz spectroscopy of remote samples. “The challenge was to control the way in which two-colour ultrafast lasers extract electrons and generate THz radiation that is sufficiently intense to reach the material to be analysed through the atmosphere, where water molecules absorb these waves,” explains Luc Bergé. In a single laser shot, the team managed to collect numerous spectral signatures at a distance of 15 m and characterise some twenty powdered samples (amino acids, sugars, explosives, drugs) with spectroscopy.
The next phase, ALTESSE 2, which will be completed in 2023, will focus on the femtosecond laser filamentation process, a non-linear optical phenomenon in which the laser beam, coupled to a plasma channel, propagates in a self-focused manner over a large distance. The team also plans to study new detection systems and propose a high-performance glass-based demonstrator for detecting explosives at more than 20 m to the French Defence Innovation Agency (AID).
Photoinjection of nanoparticles
Rachel Méallet-Renault, from the Orsay Institute of Molecular Science (ISMO – Univ. Paris- Saclay, CNRS), together with colleagues from the Nara Institute of Science and Technology (NAIST) in Japan, the Supramolecular and Macromolecular Photophysics and Photochemistry Laboratory (PPSM – Univ. Paris- Saclay, ENS Paris-Saclay, CNRS) and the Paris- Saclay Galien Institute (IGPS – Univ. Paris- Saclay, CNRS), conducted photoinjection experiments with luminescent nanoparticles (decorated with a chromophore from the BODIPY family) into living plant cells. The scientists studied the effects of a targeted 20 nJ femtosecond laser pulse on the cell membrane. “The femtosecond laser causes photoporation. The deposited energy induces local heating and a cavitation bubble which deforms the membrane and allows the nanoparticles into the cell.”
Using fluorescence imaging coupled with rapid video microscopy, the team followed the penetration and then the diffusion of nanoparticles in the cells in real time. This penetration is also observed when the laser irradiation targets a region close to the cell. While a low laser intensity threshold and a size limit of the nanoparticles are necessary to get them into the cell, the team did not observe any changes in pulse duration on diffusion.
“It has been observed that nanoparticles are also found in the cells adjacent to the irradiated cell. This opens up new insights into cell-cell interactions. By following the path of the nanoparticles, we can obtain information on the viscosity of the cell and its potential metabolic changes.” The researcher now plans to transfer the technique to bacteria. “It can be used as an alternative to electroporation, which is frequently used but is very damaging for these cells in the presence of nanoparticles.”
So, have you been convinced by the brilliance of lasers?
Publications
- A. Golinelli, et al. CEP-stabilized, sub-18 fs, 10 kHz and TW-class 1 kHz dual output Ti:Sa laser with wavelength tunability option. Opt. Express 27, (2019).
- Huijts, J., et al. Broadband coherent diffractive imaging. Nat. Photonics 14, (2020).
- Y. Wang, et al. Thermal stability of type ii modifications inscribed by femtosecond laser in a fiber drawn from a 3D printed preform. Appl. Sci. 11(2), 600, (2021).
- J. Déchard et al., Terahertz emission from submicron solid targets irradiated by ultraintense femtosecond laser pulses. Phys. Plasmas 27, 093105 (2020).
- T.I. Rukmana, et al. Direct observation of nanoparticle diffusion in cytoplasm of single plant cells realized by photoinjection with femtosecond laser amplifier. Appl. Phys. Express 13, (2020).